Designing with Silicon Carbide in Bidirectional On-Board Chargers
Article
Electric vehicle (EV) on-board chargers (OBCs) can take several forms based on power level and functions. Charging powers vary from less than 2 kW in applications such as electric scooters to 22 kW in high-end EVs. Traditionally, charging power is unidirectional. Bi-directional charging is gaining traction in the recent years. This paper will focus on bidirectional OBCs and discuss the advantages of Silicon Carbide (SiC) in both medium-power (6.6 kW) and high-power (11-22 kW) OBCs.
Why the move to bidirectional OBCs?
As the automotive world moves toward replacing gasoline with cleaner fuel alternatives, the EV transportation segment is experiencing rapid growth. As EVs operating purely on electric power continue to increase market share, the installed battery capacity per vehicle is also increasing. Consumers are also demanding faster charging times for larger-capacity batteries. This demand is also spurring an increase in battery operating voltage from 400 V to 800 V, beginning with high-performance vehicles.
An EV equipped with sufficient battery capacity is potentially capable of acting as an energy-storage system, enabling a variety of vehicle-to-everything charging use cases: vehicle-to-home power generation, vehicle-to-grid opportunities, or vehicle-to-vehicle charging. As a result, the OBC is migrating from a unidirectional to a bidirectional topology. There is a general trend toward the employment of bidirectional OBCs for their higher system efficiency.
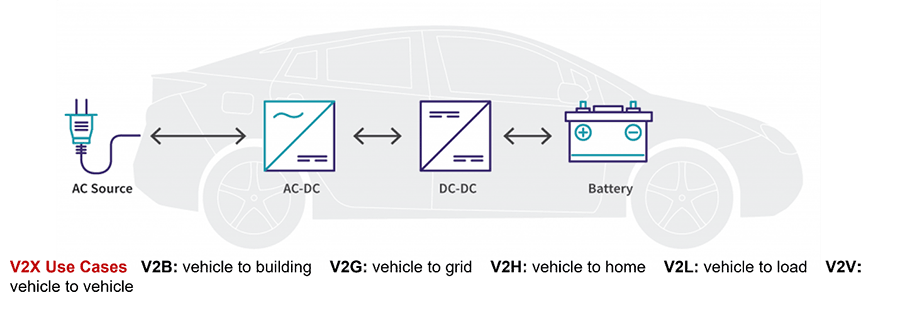
Bidirectional OBC system blocks
An OBC design for an EV requires high power density and maximized efficiency to make the most of the available EV space and minimize the weight. A bidirectional OBC consists of a bidirectional AC/DC converter, typically a power-factor–correction (PFC) or active front end (AFE) circuit followed by an isolated bidirectional DC/DC converter. Let’s examine these blocks separately.
PFC/AFE block
At the input, a conventional PFC boost converter is the most widely used topology for single-phase use, but it does not support bidirectional operation and is relatively inefficient. The totem-pole PFC improves efficiency by eliminating the bridge rectifier stage, reducing the number of semiconductor devices in the conduction path from three to two.
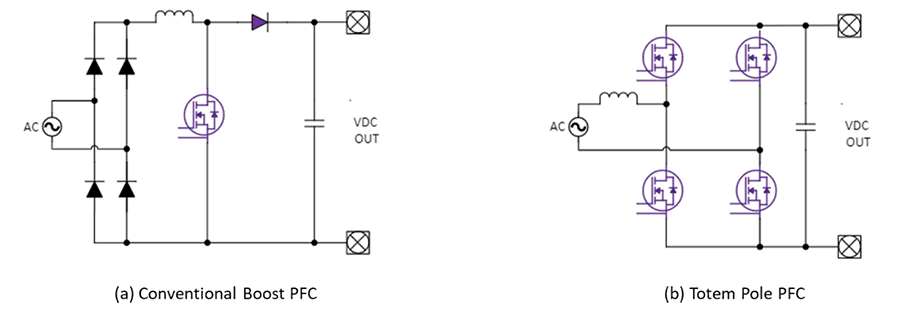
The totem-pole PFC contains two sections (legs) that operate at different frequencies. The high-speed leg in the purple box boosts the voltage and shapes the current; this leg must switch at a high frequency. The low-speed leg primarily rectifies the input voltage and can switch at the line frequency of 50/60 Hz.
In some regions in Europe, three phase power is available for residential utility, typically three phase 6 switch PFC/AFE topology can be used as shown in figure 3.
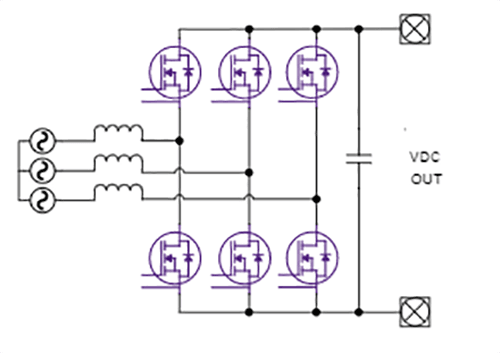
There are other types of three phase PFC such as T-type PFC, which is a 3-level converter. The benefits of the 3 level converter are lower switching loss and smaller inductor size. However, the benefits come with increased complexity of the system, more 5counts, higher total cost and overall size of the converter. So the basic 2 level three phase PFC converter shown in figure 3 is the most commonly used topology for three phase bi-directional OBC.
DC/DC converter block
The DC/DC converter in a unidirectional OBC is typically an LLC resonant converter, but this is a unidirectional topology: In reverse operation mode, the voltage gain of the converter is limited, reducing its performance. A bidirectional CLLC resonant converter in Figure 4 is therefore preferred for the DC/DC stage, as it combines high efficiency with a wide output voltage range in both charging and discharging modes.
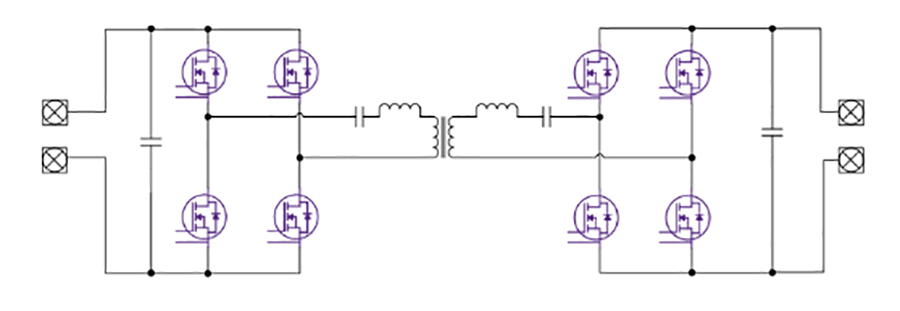
In an EV OBC application, the CLLC employs soft switching for higher efficiency: zero-voltage switching (ZVS) on the primary side and ZVS combined with zero-current switching on the secondary side.
Another common bi-directional DCDC converter topology is dual active bridge (DAB). DAB’ s operation is quite simple, regulating output by phase shifting. However, it has limited range of ZVS, and has higher switching loss than CLLC since DAB turn-off current is higher than CLLC. So, in general, DAB has lower efficiency than CLLC. On the other hand, the design of resonant circuit in CLLC is more complicated.
The many advantages of Silicon Carbide
SiC is preferred for high-power systems due to its unique combination of high critical electric field, high electron drift velocity, high temperature, and high thermal conductivity. On the transistor level, this leads to a low on-state resistance (RDS(on)) and low switching loss that makes it ideal for high-current high voltage applications.
Apart from SiC, there are two other options for the active devices in a high-power design: silicon (Si) MOSFETS and Si IGBTs. A Si MOSFET is impractical for high-power applications in the totem-pole PFC. The reverse recovery of the Si MOSFET body diode leads to high power losses in continuous-conduction mode (CCM), so its use is restricted to discontinuous-mode operation and low-power applications. In contrast, the SiC MOSFET allows the totem-pole PFC to operate in CCM for high efficiency, low EMI, and increased power density. For the voltage rating, Si MOSFET can have good Rdson performance up to 650 V. For 1200 V class, Si MOSFET’s Rdson will be too high for such high-power applications.
A SiC MOSFET also has advantages over an IGBT. The IGBT does not contain a body diode; an ultra-fast diode may be used instead. But the IGBT’s maximum switching frequency is limited due to its high switching loss. The low switching frequency increases the weight and size of the magnetics and passive components compared with a SiC solution.
Medium-power bidirectional OBC architecture (<6.6 kW)
A medium-power OBC typically operates from a single-phase 120 V or 240 V input with a 400 V DC bus. The topology is single phase totem pole PFC followed by a CLLC DCDC converter, shown in Figure 5.
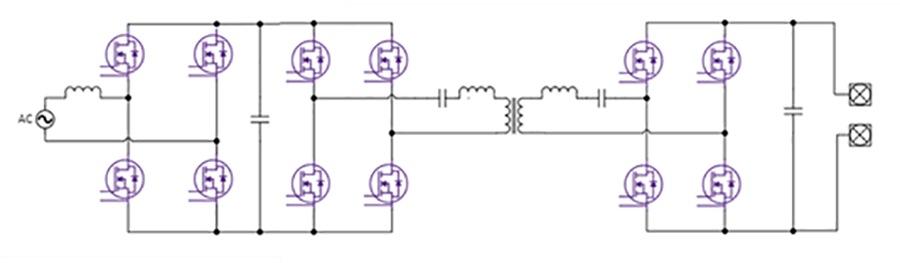
For 6.6 kW, two 60 mΩ MOSFET (e.g. E3M0060065K) in parallel or one 25 mΩ can be used for each position in PFC and one 60 mΩ (E3M0060065K) or 45 mΩ (e.g. E3M0045065K) device for each position in DCDC. Device choices for this bi-directional OBC design are summarized in following Table.
Power | SiC MOSFET Voltage Rating | SiC MOSFET PFC | SiC MOSFET DCDC |
---|---|---|---|
3.3kW | 650 V | 60mO | 120 mΩ |
6.6 kW | 650 V | 2 × 60mΩ or 25mΩ | 60 mΩ or 45 mΩ |
A 6.6 kW OBC reference design based on the architecture in figure 5 was created by Wolfspeed team to demonstrate the performance and practical use of SiC MOSFET for this application.
The table shows the requirements.
Description | Requirements |
---|---|
Input Voltage | 1Φ, 240V/120V |
Output Voltage 400V Battery | 250 - 450 Vdc |
Rated Power | 6.6 Kw (Derating r or 180 |
ACDC peak Efficiency | >98.5% |
DCDC peak Efficiency | >98% |
DC Bus Voltage | 400V |
Detailed information on the Wolfspeed 6.6 kW high power bi-directional OBC reference design can be found online.
Higher-power bidirectional OBC designs (11 kW/22 kW)
At higher power levels such as 11 kW or 22 kW, the battery voltage can be either 400 V or 800 V, but as mentioned earlier, the market is trending toward 800 V. Figure 6 shows the system block diagram for the high power three phase bi-directional OBC.
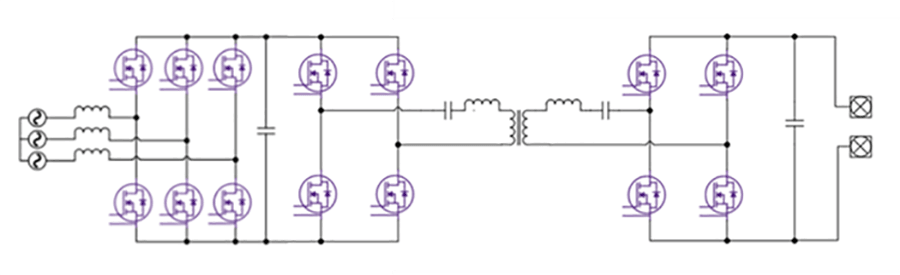
The design can accommodate either a 400 V or an 800 V bus.
The 11 kW design can use 75 mΩ 1200 V MOSFET (e.g. E3M0075120K) for PFC and the primary side of the CLLC converter. On the secondary side, an 800V battery application uses the same 75 mΩ MOSFETs as the primary. 40 mΩ 1200 V MOSFET can be used for high performance applications. For a 400 V battery application, four 650 V 25 mΩ MOSFETs can be selected for the secondary side.
The 22kW design mirrors that of the 11 kW OBC, but the higher power output requires lower Rdson devices. A 32 mΩ 1200 V MOSFET will be used for PFC and primary side of DCDC. As before, the secondary side can either use the same primary-side devices for 800 V bus applications or substitute 650 V 15 mΩ for 400 V applications.
Device choices for the high-power three-phase design are summarized in Table 3.
Power | SiC MOSFET PFC (1200 V) | SiC MOSFET DCDC Primary (1200 V) | SiC MOSFET DCDC Secondary 1200V (for 800 V battery) | SiC MOSFET DCDC Secondary 650V (for 400 V battery) |
---|---|---|---|---|
11 kW | 75 mΩ or 40 mΩ | 75 mΩ or 40 mΩ | 75 mΩ or 40 mΩ | 25 mΩ |
22 kW | 32 mΩ | 40 mΩ or 32 mΩ | 40 mΩ or 32 mΩ | 15 mΩ |
Two reference designs for 3 phase Bi-directional OBC were created by Wolfspeed, one for 22 kW three phase PFC, one for 22 kW DCDC. The table below shows the requirements for a high-power 22 kW OBC. The OBC design achieves overall efficiency greater than 96%, with DC/DC peak efficiency greater than 98.5% for both charging and discharging modes. More detailed information can be found in Wolfspeed website for the three phase 22 kW PFC and for 22 kW DC/DC.
Input Voltage | 3phase AC Input Charging | 1phse AC Input charging | Discharging Mode |
---|---|---|---|
Input Voltage | 304Vac ~ 456Vac | 90Vac ~ 277Vac | 300Vdc - 800Vdc |
Output Voltage | 200 - 800Vdc | 200 - 800Vdc | 220Vdc |
Rated Power | 22kW 36A max | 6.6kW | 6.6kW |
OBC peak Efficiency | >96% | >96% | >96% |
DCDC peak Efficiency | >98.5% | >98.5% | >98.5% |
DC Bus Voltage | 650V - 900V | 380V - 900V | 360V - 760V |
22 kW Silicon Carbide-based design with single-phase input and three phase input
In many European homes, three-phase power is readily available, but typical U.S. homes, Asian and South American homes have only a 240 V single-phase as standard. In this case, where the design calls for a high-power 22 kW OBC, which can be compatible with both single phase and three phases to reduce OBC SKU. A fourth leg is added to conventional three phase (leg) PFC such that the designer can use an interleaving technique for single phase input. Figure 7 shows an interleaved totem-pole PFC with three high-frequency sections plus the fourth low-frequency leg. The high-frequency leg of each PFC supplies 6.6 kW via 32 mΩ 1200 V SiC MOSFETs. The low-frequency leg can use two Si IGBTs to reduce cost. When three phase is available, this circuit can automatically re-configure itself to three phase operation, leaving the fourth leg floating.
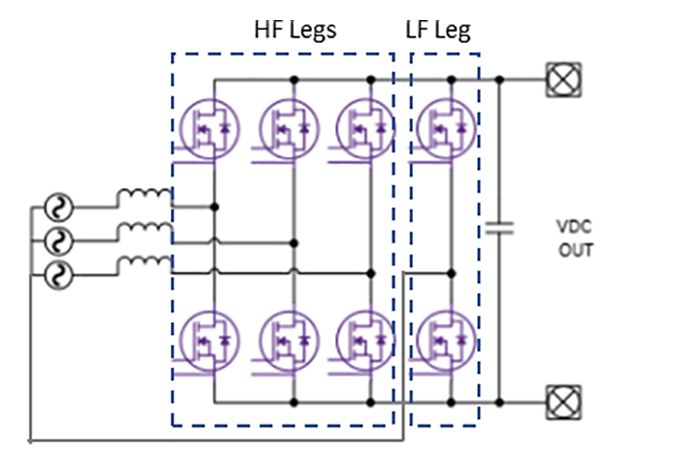
The bottom line: Silicon Carbide versus silicon in a 22 kW bidirectional OBC
In a bidirectional OBC, a SiC-based solution is superior to a Si-based solution in all relevant aspects: cost, size, weight, power density, and efficiency. In a 22kW bidirectional OBC, for example, a detailed side-by-side comparison ( Why Choose SiC over Si for Your Next Bi-directional On-Board Charger Design?) shows that the SiC-based solution shown in Figure 6 requires 14 power devices and 14 gate drivers; the Si-based design needs 22 of each type of device.
When comparing performance, the SiC design achieves 97% efficiency and a power density of 3 kW/L, versus silicon at 95% efficiency and 2 kW/L.
Finally, a system cost breakdown shows that the Si-based solution is approximately 18% more than the SiC design. A 6.6 kW comparison also shows the superiority of the SiC design, with improvements across the board.
These advantages add up to a net lifetime savings of ~$550 for the SiC system versus a Si design.
About Wolfspeed Silicon Carbide devices
Bidirectional function is the new trend in EV OBC designs. Wolfspeed SiC MOSFETs address many power-design challenges by providing devices with low on-resistance, very low output capacitance, and low source inductance for a perfect blend of low switching losses and low conduction losses. Compared with Si-based solutions, Wolfspeed SiC power-device technology enables increased system power density, higher switching frequencies, reduced component count and size of components such as inductors, capacitors, filters, and transformers, and potential system cost reduction.
This article has reviewed bidirectional designs for 6.6 kW and 22 kW OBC applications and outlined the performance advantages and cost savings that can be obtained by switching to SiC-based solutions. To learn more about Power design considerations and other topics, please visit Wolfspeed Knowledge Center.